Gene editing with CRISPR
Expert reviewers
Essentials
- CRISPR is a quick, easy and inexpensive tool for editing genes in humans, animals and plants.
- It uses a naturally occurring bacterial defence system to find, cut, edit, add or replace genes.
- Applications range from treating genetic diseases to eradicating pest species.
- When CRISPR is coupled with gene drives, genetic changes could quickly spread throughout a population.
- Rapid developments in CRISPR gene-editing technology have ethical and environmental implications.
What is this thing called CRISPR?
If you’ve been following the news in the last few years, it’s likely you’ll have heard of CRISPR (pronounced ‘crisper’). You may know that it’s got something to do with editing genes, that it was recently used for the first time to genetically alter a human embryo, and that it’s generating a lot of discussion.
Yet it may surprise you to find out that CRISPR itself is not a machine, a computer program or even a human invention—it’s actually an ancient, naturally occurring defence system. Found in a range of bacteria, CRISPR defends cells by identifying the DNA of invading viruses and, together with a protein made by the bacteria, slicing parts out of the virus to deactivate it—like a pair of DNA-cutting scissors.
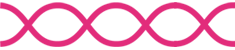



In the last few years, scientists have worked out how to harness this mechanism to cut particular sections of (non-virus) DNA and, since then, the technology has been rapidly taken up by researchers around the world for a wide range of potential applications.
Gene editing using molecular tools itself is not new (gene-editing techniques have been around for about 40 years), nor is CRISPR the only gene-editing tool currently available. But CRISPR is having a particular impact because of the ease with which it can be used, its high efficiency and its low cost.
How CRISPR works
Although usually shortened to just ‘CRISPR’, this gene-editing technology actually consists of three elements: a Cas protein, and two types of RNA. Let’s look at each of these elements, and how they function together, in more detail.
In the 1980s, scientists noted an interesting pattern in some bacterial genomes. They saw a repeating DNA sequence, which read the same forwards and backwards (that is, it was palindromic). These Clustered Regularly Interspaced Short Palindromic Repeats were abbreviated to CRISPR.
Researchers discovered that there were unique, non-repeating sequences between the repeats. And these, it turned out, matched the DNA of viruses that prey on bacteria. But how did they get there?
Well, when a virus invades, it injects its own DNA into the bacterial cell. If there is no immune response, this virus DNA hijacks the cell to produce new virus particles and, when the new viruses are released, eventually kills it.
CRISPR prevents this by employing a nifty defence mechanism. This is where Cas (which stands for CRISPR-associated) proteins come in. Cas is the ‘cutting’ part of the defence mechanism, and scientists have found that the genes that encode for Cas are always somewhere near the CRISPR sequences. There are a number of Cas enzymes, but the best known is Cas9 (it comes from Streptococcus pyogenes, the bacteria that causes ‘strep’ throat). When a virus that the cell hasn’t encountered before attacks, Cas breaks the virus’s DNA and takes it into the CRISPR locus. These bits of virus DNA are the non-repeating sequences observed by scientists. Put simply, the bacterial cell keeps bits of the invading virus around so it can recognise it if the virus attacks again.
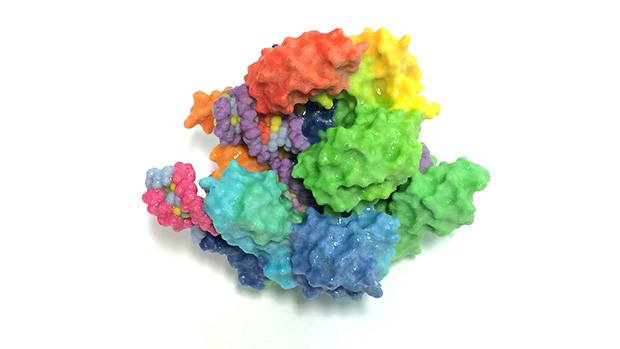
So that it can recognise later infections with the same virus, the bacteria assembles a few bits and pieces in its virus-busting toolkit. First, it copies the entire CRISPR locus containing the bits of viral DNA into a long RNA. The long RNA is then chopped up into smaller pieces called CRISPR RNAs. These are individually taken up by Cas proteins, together with another kind of RNA, known as trans-activating RNA. This useful new combo is then ready to survey the cell for invading viral DNA.
The next time a virus with DNA matching one of the non-repeating sequences in the CRISPR invades, the system recognises it. The RNA complex held by the Cas protein binds to the matching viral DNA, so the RNA can instruct the Cas protein exactly where to cut. This complex snips both strands of the virus DNA, inactivating the gene and preventing the virus from infecting the cell.
This knowledge has revolutionised the way scientists can modify genes. In 2012 (following the work of several groups before them), the teams of two remarkable women, Emmanuelle Charpentier (at Umea University, Sweden, at the time) and Jennifer Doudna (University of California, Berkeley), came up with a way of using this clever bacterial defence strategy to cut any DNA sequence and edit genomes. Now, once scientists know what sequence of DNA they want to cut, all they have to do is insert the appropriate guide RNA into the Cas protein. The Cas protein will follow these ‘instructions’ and cut the DNA at the specified place. In this way, scientists can ‘edit out’ an undesirable gene.
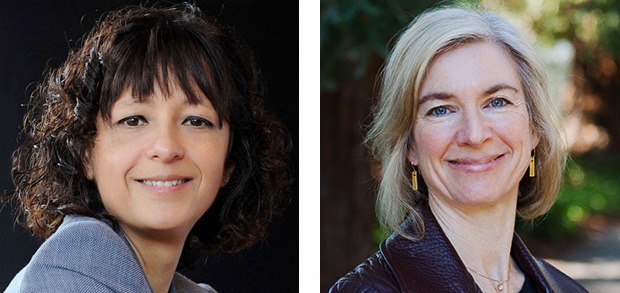
Not only does the CRISPR-Cas9 system allow scientists to ‘delete’ genes, but also to replace faulty genes carrying disease-causing mutations with intact ones. When the DNA strand is broken, the cell’s repair systems kicks in to repair the break. Scientists can take advantage of this process by inserting new sequences of DNA at the repair site, thereby changing the gene sequence.
Refining the technology
CRISPR-Cas9 is not yet a perfect, or risk-free, technology. One problem still to be resolved is ‘off-target’ editing: that is, where Cas9 acts at sites other than the one targeted. While the current error rate of Cas9 is probably adequate for most research applications, the accuracy of the enzyme needs to be improved before it can be used therapeutically.
Researchers have been looking at various ways to do this, including tweaking the guide RNA and looking at ways of switching off the system. Several labs around the world, including Keith Joung at the Massachusetts General Hospital and Feng Zhang and his team at the Broad Institute of MIT and Harvard in Cambridge, have been creating new versions of Cas9 which significantly reduce off-target errors, although they may still not be accurate enough for therapeutic use. A further issue is what’s known as ‘mosaicism’. In cases where CRISPR technology is applied to multicellular organisms, there is the possibility that only some of the cells will be edited, resulting in a mixture of edited and unedited cells.
Work is also being done on a new system that may be even simpler than CRISPR-Cas9, with recent research by Charpentier demonstrating that a protein called Cpf1 may do the work of both the trans-activating RNA and the Cas9 protein.
Editing the genome: everything from disease models to modified mozzies
A cure for genetic diseases? A slippery slope towards designer babies? These are some of the headline-grabbing claims that have been made about gene editing with CRISPR. But the applications of this technology are actually much more wide ranging—from enabling new kinds of laboratory research to eradicating pest species.
Creating model organisms for research
Model organisms are those that can be used to study various processes and diseases in the lab—because they reproduce quickly and are easily genetically manipulated, mice and fruit flies have traditionally been used for this purpose. CRISPR, however, enables scientists to more easily carry out genetic research across a wider range of organisms. Researchers at the Whitehead Institute for Biomedical Research, Massachusetts, for instance, recently reported using CRISPR to study Candida albicans, a potentially deadly yeast which has previously been difficult to manipulate in the laboratory.
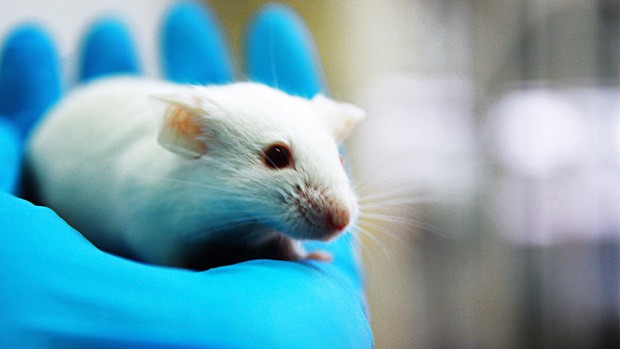
Treating disease with gene therapy
In 2014, researchers at MIT were the first to use CRISPR to treat a disease-causing mutation in an adult animal. In humans, the mutation causes a metabolic disease called tyrosinaemia. The work, carried out in mice, was not without problems, however. A large amount of liquid had to be pumped into blood vessels (probably not feasible in people) and only a small percentage of the cells were corrected. CRISPR-Cas9 has also been successfully used to improve muscle function in mice suffering from a disease similar to Duchenne muscular dystrophy, a genetic muscle disease.
CRISPR has also recently been used to edit HIV from T cell genomes in human cells (in the lab) by introducing mutations into the viral genome. HIV works by inserting its genome into the host cell’s DNA. While antiretroviral drugs keep active infection at bay, the viral DNA rebounds if a patient stops taking them. Editing the virus’s genome, by contrast, permanently prevents it from replicating. While the research found no off-target effects or adverse consequences of the gene treatment for cell health, another team reported that HIV can become ‘resistant’ to gene editing.
So, while an exciting prospect, it is clear that much more work is needed before human diseases can be safely treated using CRISPR.
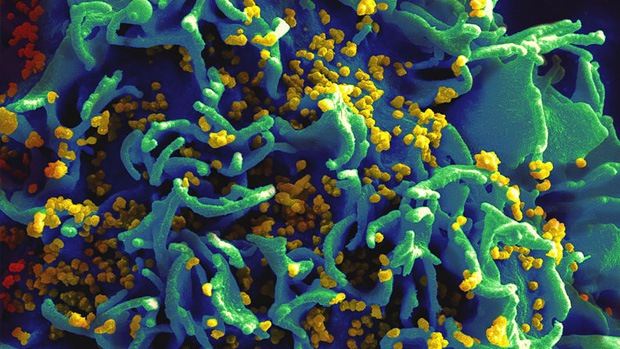
Altering crops and livestock
Gene editing of crops and livestock using CRISPR is another area of research. While traits have long been modified through selective breeding, often coupled with genetic change techniques such as chemical mutagens or recombinant DNA technology, CRISPR may prove to be a quicker, easier and more accurate method. Scientists in China, for instance, have been looking into developing goats with longer coats (for angora) and more muscles (for increased meat yield), while disease-resistant wheat and rice have also been developed in the lab.
The editing of crops and livestock using this new technology is proving to be a particular challenge for regulation. Genetically modified organisms are currently covered by regulations which apply to cases in which new genetic material has been introduced. But, because CRISPR is often used to change the DNA of an organism without introducing new genetic material, it’s unclear how this new technology will be regulated by the existing system.
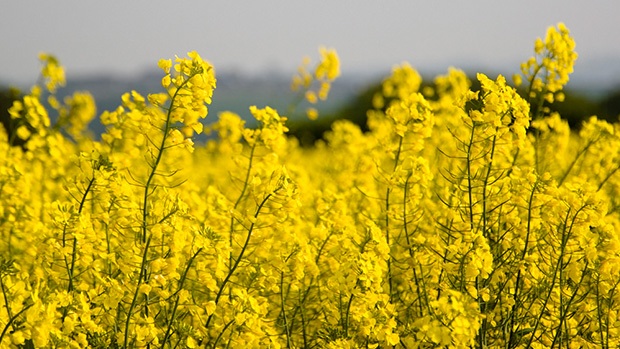
Modifying animal organs for transplantation
CRISPR may also have a role to play in the modification of animal organs for transplantation into humans. The tissues and organs of pigs are generally considered a good option for transplants into humans—they are readily available, and their organs are a similar size to ours. However, the transplantation of pig organs and tissues into humans not only carries the risk of rejection, but may introduce endogenous retroviruses (found in the pig genome) into the human body, with the potential for serious infection if activated. By genetically modifying the pig genome, these problems could be eliminated—giving the patient more time while they wait for a human organ, or enabling the pig organ to function as a permanent transplant. While Harvard scientists have used CRISPR-Cas9 to target pig genes that trigger an immune response in people, there are still challenges to be addressed—such as the possibility that unknown pathogens in the donor organ could be introduced to the recipient.
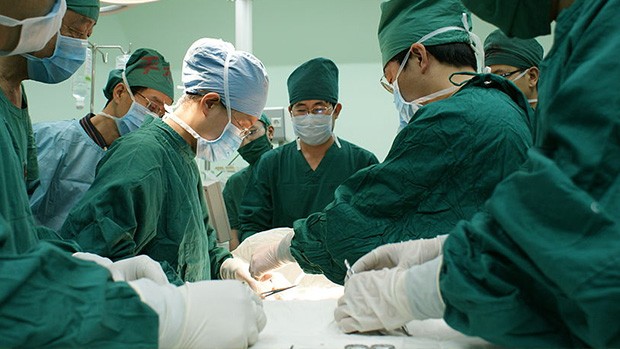
Eradicating animal-borne disease
An important area of research is into the use of CRISPR to eradicate disease spread by animals, such as mosquito-borne malaria, tick-borne Lyme disease or schistosomiasis, a disease caused by parasitic trematode worms. Scientists have been looking closely at how they might edit the genome of malaria-carrying mosquitoes as an alternative to treating people with anti-malaria drugs (to which the malaria parasite can evolve resistance). In work at the University of California, Irvine, anti-malarial genes were introduced into the DNA of mosquito eggs, effectively creating modified mozzies which can’t pass malaria on to humans. By coupling CRISPR with gene drives (more below), the idea is that mosquitoes modified in the lab could breed with wild mosquito populations, quickly spreading the anti-malaria gene.
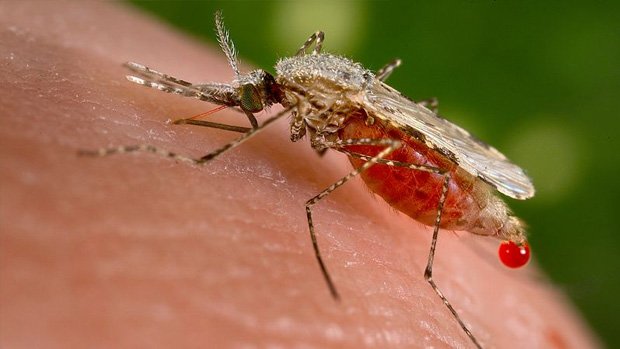
Human germline editing
Perhaps the most controversial use of the new technology, and one you’ll likely have heard about in the media, has been the modification of human sperm, eggs or early embryos, known as germline editing. In contrast to the editing of somatic (non-reproductive) cells, germline editing results in heritable changes—that is, genetic changes that don’t just happen in the individual organism, but are passed down to future generations.
In 2015, Chinese scientists generated controversy when they published a paper reporting that they had attempted genetic editing using CRISPR on (donated, non-viable) human embryos. While CRISPR had been used to modify the genomes of animal embryos and human adults, this was the first time the genome of human embryos had been edited. The experiments were not very successful, with a number of off-target effects and instances of mosaicism. The researchers concluded, in a marked understatement, that clinical use of CRISPR to edit embryos was ‘premature at this stage’.
The prospect of human germline editing using CRISPR in the future has raised a number of moral and ethical questions among scientists and the general public, including:
- Given the relative ease with which it can be used, its cheapness, and the patchwork nature of current laws relating to gene editing across the globe , how can the use of CRISPR be effectively regulated?
- Should germline editing be allowed for clinical use, or only for basic research purposes in the lab?
- If clinical use is allowed, should it be only for medically justifiable, but not ‘non-essential’, alterations to the human genome? And where do we draw the line between what is ‘justifiable’ or not?
- Should it be acceptable to create embryos specifically for research purposes?
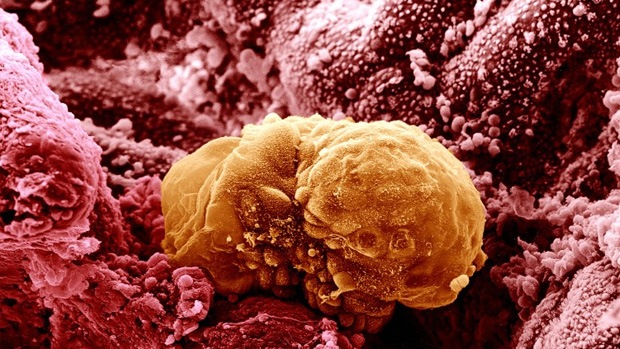
Spreading genetic changes with CRISPR gene drives
Making a genetic change in an individual organism is one thing. But what if, as in the case of pest or disease-carrying species, we want a particular change (such as the cutting out of a gene in mosquitoes which transmits the malaria parasite) to spread throughout a whole population? In this case, the challenge is to introduce a genetic disruption in such a way that it has a high chance of being transmitted to every generation.
In organisms that have two parents—and two sets of chromosomes—there is only around a 50-50 chance of a particular gene being passed down to offspring. But CRISPR can now be used as a new form of ‘gene drive’ (that is, genes which give themselves a 100 per cent chance of being passed on by making sure they show up on both chromosomes in a pair) to increase those odds.
Through standard inheritance, offspring have a 50 per cent chance of inheriting a modified gene carried by one of their parents. Over time, genes spread slowly through the population.
Modified from CRISPR, the disruptor (Nature)
An organism with a gene drive mechanism can modify the chromosomes passed on by its partner during reproduction. It does this by cutting the partner's chromosome and inserting the modified gene. When paired with a gene drive mechanism, modified genes are inherited by almost 100% of offspring, allowing the gene to spread rapidly through the population.
Modified from CRISPR, the disruptor (Nature)
In the case of CRISPR-Cas9, the gene drive ‘construct’ consists of the Cas9 protein, plus the Cr-TrRNA (the CRISPR RNA together with a small trans-encoded RNA), flanked by two long sequences identical to the gene (let’s call it Gene X) we want to target. Because of the stretch of homology (similarity) with the target gene, the CRISPR construct first integrates itself into that gene. By doing so, it interrupts the gene’s normal sequence, making it non-functional.
Next, the product of this CRISPR construct recognises and cuts Gene X in the other chromosome. The cell then repairs this DNA break by using the gene-drive CRISPR-Cas9 construct as a template. Hey presto—the gene drive has now been copied onto the second chromosome.
Now both chromosomes lack an intact Gene X, and both contain identical CRISPR sequences. When the cell divides, splitting its chromosomes, both new cells will end up with a copy of the gene drive. What this means is that, even if only one parent has the gene drive, it will end up in all the offspring. Over a number of iterations of this process, the entire population will end up with the gene drive.
By using CRISPR as a form of gene drive, specific genes can be spread through a population. In the case of mosquitoes, for example, this could be a desired trait (like an anti-malaria gene) or a ‘deleterious gene’ (one that will lead to the demise of the mosquito population). Because it relies on a quick succession of generations to spread the gene change, the use of gene drives is only practical in species which have a short generation time—making organisms such fruit flies and mosquitoes (but not vertebrates) perfect candidates.
While it may have enormous potential for controlling pests and diseases, there are serious concerns about the risks of using CRISPR-engineered gene drives in wild animal populations. Mating between two species could mean that a gene-driven mutation hops onto an unintended species, driving it to extinction. Changes made in species in one part of the world could spread to another. And altering or wiping out whole species could have far-reaching and unpredictable ecological effects. A number of scientists have called for strict safeguards to prevent modified organisms from escaping from the lab, and looking at ways to reverse changes made with CRISPR-engineered gene drives.
Outcry over designer babies and precision gene therapy should not blind us to a much more pressing problem: the increasing use of CRISPR to edit the genomes of wild animal populations. Unless properly regulated and contained, this research has the potential to rapidly alter ecosystems in irreversible and damaging ways.Jeantine Lunshof, bioethicist
Keeping up with the technology
From the treatment of genetic diseases to ground-breaking new laboratory research, CRISPR promises some exciting possibilities. But it also comes with significant risks, as well as posing some sticky ethical and scientific dilemmas. A number of meetings around the globe are bringing together scientists and other experts to thrash out the issues. The rapid developments in this area mean that the questions raised by gene-editing technologies in general, and CRISPR in particular, need to be addressed soon—and at an international level—to determine a responsible way forward, but there is little doubt that CRISPR will prove to be a very useful research tool in the lab.